Catalisadores à base de platina em vários suportes de carbono e polímeros condutores para aplicações em células de combustível de metanol direto:uma revisão
Resumo
Os metais das nanopartículas à base de platina (Pt) têm recebido bastante atenção e são os catalisadores mais populares para células a combustível de metanol direto (DMFC). No entanto, o alto custo dos catalisadores de Pt, a oxidação cinética lenta e a formação de moléculas intermediárias de CO durante a reação de oxidação do metanol (MOR) são os principais desafios associados aos catalisadores de Pt de metal único. Estudos recentes estão se concentrando no uso de ligas de Pt, como metais de Fe, Ni, Co, Rh, Ru, Co e Sn, ou materiais de suporte de carbono para melhorar o desempenho catalítico de Pt. Nos últimos anos, os catalisadores de liga de Pt e Pt apoiados em grande potencial de materiais de carbono, como MWCNT, CNF, CNT, CNC, CMS, CNT, CB e grafeno têm recebido interesses notáveis devido às suas propriedades significativas que podem contribuir para o excelente MOR e desempenho de DMFC. Este artigo de revisão resume o desenvolvimento das ligas acima e materiais de suporte relacionados para reduzir o uso de Pt, melhorar a estabilidade e melhor desempenho eletrocatalítico de Pt em DMFC. Finalmente, a discussão de cada catalisador e suporte em termos de morfologia, atividade eletrocatalítica, características estruturais e seu desempenho de célula de combustível são apresentados.
Introdução
A tecnologia de células de combustível ganhou grande atenção em todo o mundo. As células a combustível (FCs) são uma alternativa promissora de tecnologia de geração de energia que converte energia química em energia elétrica por meio de uma reação eletroquímica [1, 2]. Além disso, para a tecnologia de célula de combustível, o foco principal na tecnologia de célula de combustível é gerar produção de baixo custo, alcançando assim um desempenho poderoso do sistema de célula de combustível e descobrindo materiais duráveis. No entanto, os problemas comuns que surgem na tecnologia atual de células de combustível são que os sistemas envolvem altos custos intrínsecos e pouca durabilidade [1]. Apesar de sua promessa como uma célula a combustível, as células a combustível de metanol direto (DMFCs) têm desafios e limitações, levando os pesquisadores a estudar métodos para melhorar a eficiência e o desempenho do DMFC. Muitos problemas com DMFCs foram identificados e permanecem sem solução, incluindo cruzamento de metanol combustível do eletrodo anódico para o eletrodo catódico [3,4,5] desempenho insatisfatório causado pela taxa de cinética lenta, instabilidade do catalisador e gerenciamento térmico e de água [6,7,8].
Recentemente, houve inúmeras investigações sobre células de combustível, incluindo DMFC, célula de combustível de membrana de troca de prótons (PEMFC), célula de combustível de óxido sólido (SOFC) e assim por diante, que são tecnologias de células de combustível populares. Como uma nova fonte de energia, os DMFCs podem ser usados para aplicações móveis e estacionárias [9, 10]. Muitos avanços de pesquisa foram alcançados no campo das células de combustível. Entre as células a combustível, as DMFCs têm sido amplamente estudadas nos últimos anos [11,12,13,14,15,16] devido às suas muitas vantagens, como alta densidade de potência, facilidade de manuseio de combustível, facilidade de carregamento e baixo ambiente impacto [17, 18]. No entanto, vários desafios técnicos para a comercialização de DMFCs permanecem sem solução, incluindo crossover de metanol, baixas taxas de reação química e envenenamento de catalisador. No entanto, as DMFCs ainda têm recebido atenção de muitos pesquisadores e se tornaram as células de combustível mais populares devido à sua operação em baixa temperatura (os sistemas DMFC operam a 373 K). Devido às vantagens do DMFC de alta eficiência energética e sistema de inicialização rápida, a tecnologia DMFC é muito adequada para ser aplicada como fontes de energia residenciais, baterias em dispositivos móveis e como combustível de veículos [19,20,21,22]. Além disso, o conceito de DMFCs poderia ser mais estudado para encontrar fontes alternativas de combustível, como o gás natural e biomassa, bem como a fermentação de produtos agrícolas para a produção de etanol, a fim de minimizar a dependência de fontes inseguras de energia [14].
No DMFC, o lado do ânodo é fornecido com solução de metanol que sofrerá eletrooxidação em dióxido de carbono (CO 2 ) por meio da reação abaixo:
$$ {\ mathrm {CH}} _ 3 \ mathrm {OH} + {\ mathrm {H}} _ 2 \ a {\ mathrm {CO}} _ 2 + 6 {\ mathrm {H}} ^ {+} + 6 { \ mathrm {e}} ^ {\ hbox {-}} $$ (1)
Enquanto no lado do cátodo o próton, o oxigênio (do ar) é reduzido a água:
$$ 3/2 \ {\ mathrm {O}} _ 2 + 6 {\ mathrm {H}} ^ {+} + 6 \ {\ mathrm {e}} ^ {\ hbox {-}} \ a 3 {\ mathrm {H}} _ 2 \ mathrm {O} $$ (2)
A reação DMFC da equação líquida pode ser resumida da seguinte forma:
$$ {\ mathrm {CH}} _ 3 \ mathrm {OH} +3/2 {\ mathrm {O}} _ 2 \ a {\ mathrm {CO}} _ 2 + 2 {\ mathrm {H}} _ 2 \ mathrm { O} $$ (3)
Em sistemas DMFC, existem dois tipos de modos DMFC:modos ativo e passivo [23,24,25]. Em um sistema DMFC ativo, o fluxo de saída da pilha de DMFC é recirculado através do controle de circuito fechado da alimentação de metanol líquido. Enquanto isso, o metanol líquido na corrente do ânodo é controlado por um sensor de concentração de metanol que desempenha um papel importante no fornecimento de injeção suficiente de metanol e água adicionais para restaurar este combustível com base na concentração alvo. Existem vários tipos de sensores de concentração de metanol que são usados no sistema DMFC para controlar e manter a concentração de metanol na alimentação [17]. Normalmente, o metanol líquido é fornecido ao lado do ânodo por uma bomba peristáltica, enquanto o ar circundante contendo oxigênio é fornecido ao lado do cátodo por um soprador ou ventilador [16]. Em um modo passivo do sistema DMFC, o metanol líquido é alimentado continuamente ao sistema. Este conceito passivo é muito atraente para sistemas DMFCs [26,27,28]. O conceito de passivo significa que o sistema opera de forma totalmente autônoma, sem quaisquer dispositivos de suporte. O conceito de DMFC passivo significa que o sistema opera de forma totalmente autônoma, sem qualquer auxílio de dispositivo externo para bombear metanol e soprar ar na chaminé. No modo passivo do sistema DMFC, a camada de catalisador será fornecida por metanol e oxigênio como reagentes. Durante a reação de oxidação do metanol (MOR), CO 2 e a água será removida da célula por meios passivos, ou seja, difusão, convecção natural, ação capilar, etc. [20]. O modo DMFC passivo parece mais vantajoso em comparação com o modo ativo DMFC em termos de design mais simples e compacto e de baixo custo. O projeto e os controles do sistema complexo podem ser uma desvantagem do modo ativo DMFC [21]. Do ponto de vista dos usos práticos, o modo ativo DMFC parece ser mais adequado em sistemas de alta potência, enquanto o modo passivo DMFC é mais adequado para ser usado em requisitos de baixa potência [22].
A Figura 1 mostra a configuração e o projeto de um DMFC de célula única. Uma pilha de DMFC de célula única consiste em um conjunto de eletrodo de membrana de cinco camadas (MEA) ensanduichada por duas placas que são ânodo e cátodo. No lado do ânodo, o metanol líquido (contém metanol e água desionizada) e o metanol absoluto é fluído para o canal por uma bomba peristáltica. No lado do cátodo, o ar é bombeado para a célula de combustível por um rotâmetro. O controlador de temperatura na pilha DMFC é usado para manter a temperatura de trabalho na célula por um dispositivo de aquecimento suplementar. O dispositivo de carga eletrônica é usado para alterar a densidade da corrente para diferentes níveis e medir os valores correspondentes da tensão. O desempenho da célula é monitorado por uma estação de trabalho eletroquímica, enquanto a produção de CO 2 como produto final da reação geral é medido por um CO 2 detector de concentração [23]. Na célula a combustível de metanol direto, há vários parâmetros operacionais importantes que devem ser considerados durante o estudo experimental, que são (i) temperatura de trabalho, (ii) concentração de metanol e (iii) taxas de fluxo de entrada de solução de metanol de alimentação e ar [23] . A Figura 2a, b mostra o modo ativo e passivo do DMFC, respectivamente.
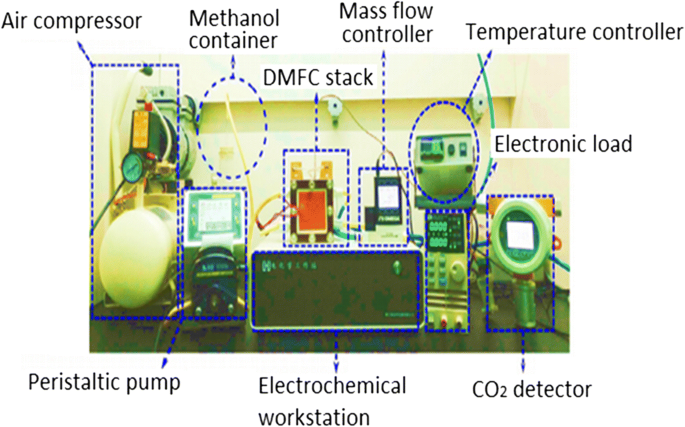
Uma configuração experimental geral para DMFC de célula única [23]
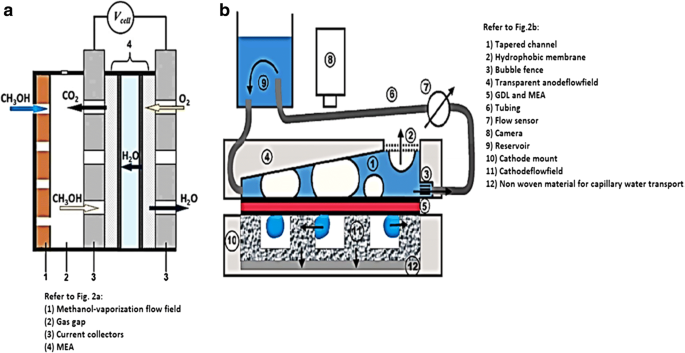
Diagrama esquemático de a modo ativo [24] e b modo passivo [25] de DMFC
Esta revisão enfocará o progresso recente nas pesquisas e desenvolvimentos de suporte de catalisador com base no catalisador Pt como o catalisador nobre em DMFC. Incluímos a atividade de catalisadores à base de Pt combinados com ligas, metais, metais de transição, carbonetos metálicos, nitretos metálicos e várias espécies carbonáceas, como grafeno / óxido de grafeno (G / GO), nanotubo de carbono (CNT), nanofibra de carbono ( CNF), nanobra de carbono (CNC), negro de fumo (CB), nanotubo de carbono de parede múltipla (MWCNT) e suportes de carbono mesoporoso (CMS), bem como polímeros condutores, como polianilina (PANi) e polipirrol (Ppy) como suporte material. Muitos métodos de síntese podem ser aplicados para preparar catalisadores à base de Pt. Os métodos mais comuns aplicados para obter partículas de Pt em nanoescala são impregnação [29,30,31,32,33,34], técnicas hidrotermais [35,36,37,38,39,40,41], microemulsão [42,43, 44,45] e redução [46, 47]. Geralmente, o método de preparação pode afetar a morfologia e o tamanho das partículas do catalisador; assim, a seleção do método para a síntese do catalisador é muito importante.
Desempenho de vários tipos de catalisadores baseados em Pt
Na última década, muitos pesquisadores concentraram suas pesquisas no desenvolvimento de eletrocatalisadores, a fim de aumentar sua atividade eletrocatalítica em metanol MOR para o sistema DMFC [37, 38]. Platina (Pt) é um catalisador de metal único que mostra atividade catalítica significativamente alta para o MOR. No entanto, Pt puro sozinho em um sistema DMFC pode ser facilmente envenenado pelas espécies intermediárias, ou seja, monóxido de carbono (CO), e o alto custo do catalisador de Pt limita sua aplicação comercial como eletrocatalisador, reduzindo assim a taxa cinética de oxidação do metanol no sistema DMFC [48,49,50]. Esses três pontos são os principais obstáculos e limitações do uso de Pt sozinho como eletrocatalisador para DMFC. No entanto, para superar esses obstáculos, vários estudos foram conduzidos para sintetizar eletrocatalisadores de liga à base de Pt para alcançar melhor desempenho eletrocatalítico com menos uso de Pt [11, 47, 51, 52]. Normalmente, o tamanho médio das partículas de Pt e sua morfologia podem ser determinados por meio de análise de micrografia de emissão de varredura (SEM) ou micrografia eletrônica de transmissão (TEM), que são os métodos mais comuns no campo de catálise que podem ser usados para caracterizar as propriedades físicas dos eletrocatalisadores. A Tabela 1 mostra os tamanhos médios de partícula das partículas de Pt com diferentes métodos de síntese, propriedades e seus desempenhos.
O PtRu bimetálico é considerado o catalisador mais ativo devido ao seu mecanismo bifuncional e aos efeitos do ligante [48, 53]. PtRu torna-se uma liga catalisadora interessante, e tem sido usada até hoje com diversos suportes de carbono. No entanto, o efeito toxicológico da adição de metal rutênio (Ru) permanece incerto [49]. Portanto, pesquisas sobre ligas menos caras que misturam Pt com outros metais não preciosos foram realizadas [49,50,51,52, 54,55,56,57], conforme discutido na seção “Desempenho de ligas à base de Pt”.
Desempenho de ligas baseadas em Pt
Arico et al. [35] descobriram que vários estudos foram conduzidos para aumentar a atividade catalítica dos catalisadores de Pt no MOR. Em muitos estudos, a razão Pt-Ru ideal foi identificada como 1:1, e os tamanhos de partícula na escala nanométrica são o tamanho ideal para melhorar a utilização do catalisador. No entanto, Shi et al. [38] identificaram que 3:2 era a proporção ideal para Pt-Ru em seus experimentos para aumentar a atividade catalítica de MOR. Fora isso, a atividade eletrocatalítica para a atividade de eletrooxidação do metanol também pode ser aumentada se as partículas do eletrocatalisador PtRu forem partículas nanométricas na faixa de 2–4 nm. Paulas et al. [39] concordou com esta afirmação. Como sabemos, o Pt mostra alta reatividade em relação ao combustível metanol, tornando o metal Pt um eletrocatalisador ideal para o eletrodo anódico no sistema DMFC. No entanto, durante a MOR do catalisador de Pt, o monóxido de carbono (CO), ou seja, a espécie intermediária, se formará na superfície das partículas de Pt, envenenando assim a superfície do catalisador [58,59,60,61]. Assim, alguns esforços são necessários para contornar o problema relacionado à formação de espécies venenosas na superfície das partículas de Pt, de forma que não encobram as áreas do sítio ativo de Pt. Geralmente, ligas binárias, como PtRu [62,63,64,65,66], PtRh [67,68,69,70,71], PtAu [72,73,74], PtSn [62, 63, 75, 76,77], PtNi [64,65,66,67,78,69], PtCo [70, 71, 78,79,80] e PtFe [81,82,83,84,85], são frequentemente empregados como combinações de eletrocatalisador para o eletrodo anódico no sistema DMFC. Acredita-se que a adição desses metais, como rutênio (Ru), estanho (Sn) e ródio (Rh), produza maior atividade catalítica.
A incorporação de níquel (Ni) no catalisador à base de platina oferece desempenho superior para MOR e DMFC. Em pesquisas mais recentes, Guerrero-Ortega e colaboradores explicam que a adição de Ni no suporte Pt-Vulcan promove um incremento importante na corrente farádica durante MOR de uma ordem de magnitude, embora o uso de Pt seja menor no catalisador bimetálico [ 55]. Seus resultados experimentais também sugeriram que a adição de Ni promove algumas modificações estruturais e eletrônicas que melhoram o desempenho da reação na interface do eletrodo. Em outro trabalho, a incorporação de Au na liga de Pt potencializou as atividades eletrocatalíticas devido à mudança na estrutura eletrônica e à melhoria da área eletroquimicamente ativa (ECSA) [47]. Já a adição de Estanho (Sn) à liga à base de Pt mostrou um incremento na atividade eletrocatalítica, que é fortemente influenciada pela incorporação de Sn em seu sistema de liga e formas oxidadas, potencializando a reação mais prontamente devido ao menor potencial de oxidação [ 56]. Além disso, a adição de cobalto (Co) à liga à base de Pt melhorou muito as propriedades catalíticas pelo catalisador PtCo (1:9) / rGO que se descobriu ser dez vezes maior do que Pt / rGO [51]. O aumento na densidade de corrente é atribuído à maior dispersão de nanopartículas de PtCo na natureza hidrofílica do suporte rGO que promove a ativação da água e leva a oxidar o CO ads em sites Pt. Além disso, de acordo com o mecanismo bifuncional de Co, promove o H 2 Ativação O criando mais íons -OH e outros O 2 - contendo espécies para oxidar espécies intermediárias de CO no local Pt [57]. Este mecanismo bifuncional de Co também pode ser usado para outros metais de transição catalítica para MOR. O mecanismo de oxidação catalítica para espécies de CO em CO 2 na presença de catalisadores PtCo podem ser resumidos da seguinte forma:
$$ \ mathrm {Pt} + {\ mathrm {CH}} _ 3 \ mathrm {OH} \ to \ mathrm {Pt} \ hbox {-} {\ mathrm {CO}} _ {\ mathrm {ads}} + 4 {\ mathrm {H}} ^ {+} + 4 {\ mathrm {e}} ^ {\ hbox {-}} $$ (4) $$ \ mathrm {Co} + {\ mathrm {H}} _ 2 \ mathrm {O} \ to \ mathrm {Co} {\ left (\ mathrm {OH} \ right)} _ {\ mathrm {ads}} + {\ mathrm {H}} ^ {+} + {\ mathrm {e }} ^ {\ hbox {-}} $$ (5) $$ {\ mathrm {PtCO}} _ {\ mathrm {ads}} + \ mathrm {Co} {\ left (\ mathrm {OH} \ right) } _ {\ mathrm {ads}} / {\ mathrm {CO}} _ 2+ \ mathrm {Pt} + \ mathrm {Co} + {\ mathrm {H}} ^ {+} + {\ mathrm {e}} ^ {\ hbox {-}} $$ (6)
Além disso, Löffler et al. [86] sintetizou com sucesso PtRu como o catalisador anódico para DMFCs pelo qual produziu o eletrocatalisador mais ativo para eletrooxidação de metanol em aproximadamente 50 at.% Ru. Enquanto isso, Dinh et al. relataram [87] que PtRu com proporção de PtRu 1:1 tem um comportamento metálico mais forte e maior atividade eletrocatalítica para oxidação de metanol (MOR). O desempenho está relacionado a estes dois fatores principais:(i) área de superfície maximizada do catalisador e (ii) superfície do catalisador com número máximo de sítios de liga metálica de razão atômica próxima a 1:1. Ambos os grupos também mostraram alta. Com base no mecanismo bifuncional, Aricò et al. [58] e Goodenough et al. [62] sugeriu que as espécies intermediárias de CO que se formaram nos sítios tensoativos de Pt podem ser oxidadas a dióxido de carbono (CO 2 ) por átomos de oxigênio ativos formados nos elementos secundários, por exemplo Ru, Sn e Mo, na região de potencial inferior. A Tabela 1 resumiu o desempenho de vários tipos de catalisador de liga de Pt realizado por pesquisadores para o MOR. De acordo com o mecanismo bifuncional [88,89,90], o MOR em catalisadores de liga de PtRu com suporte pode ser resumido como a seguinte equação. Pt é um catalisador mais ativo para a adsorção de metanol do que Ru. Portanto, a reação geral nos eletrocatalisadores PtRu para a reação de oxidação do metanol obedece ao mecanismo bifuncional.
$$ \ mathrm {Pt} + {\ mathrm {CH}} _ 3 \ mathrm {OH} \ to \ mathrm {Pt} \ hbox {-} {\ mathrm {CH}} _ 3 \ mathrm {OH} \ mathrm {ads } \ to \ mathrm {Pt} \ hbox {-} {\ mathrm {CO} \ mathrm {H}} _ {\ mathrm {ads}} \ to 3 \ mathrm {H} +3 \ mathrm {e} \ hbox {-} \ to \ mathrm {Pt} \ hbox {-} {\ mathrm {CO}} _ {\ mathrm {ads}} + {\ mathrm {H}} ^ {+} + {\ mathrm {e}} ^ {\ hbox {-}} $$ (7) $$ \ mathrm {Ru} + {\ mathrm {H}} _ 2 \ mathrm {O} \ to \ mathrm {Ru} \ hbox {-} {\ mathrm { OH}} _ {\ mathrm {ads}} + {\ mathrm {H}} ^ {+} + {\ mathrm {e}} ^ {\ hbox {-}} $$ (8) $$ \ mathrm {Pt } \ hbox {-} {\ mathrm {CO} \ mathrm {H}} _ {\ mathrm {ads}} + \ mathrm {Ru} \ hbox {-} {\ mathrm {OH}} _ {\ mathrm {ads }} \ to \ mathrm {Pt} + \ mathrm {Ru} + {\ mathrm {CO}} _ {2+} 2 {\ mathrm {H}} ^ {+} + 2 {\ mathrm {e}} ^ {\ hbox {-}} $$ (9) $$ \ mathrm {Pt} \ hbox {-} {\ mathrm {CO}} _ {\ mathrm {ads}} + \ mathrm {Ru} \ hbox {-} {\ mathrm {OH}} _ {\ mathrm {ads}} \ to \ mathrm {Pt} + \ mathrm {Ru} + {\ mathrm {CO}} _ 2 + {\ mathrm {H}} ^ {+} + { \ mathrm {e}} ^ {\ hbox {-}} $$ (10)
Referindo-se a este mecanismo bifuncional, o metanol é inicialmente dissociado e adsorvido em Pt, posteriormente decomposto em CO ads e / ou espécies semelhantes a formil -CHO anúncios por reação de desidrogenação (7). Ao mesmo tempo, a água se dissocia dos anúncios OH e adsorvido em sítios Ru (8). Em seguida, as espécies são adsorvidas em locais Pt e Ru e se combinam para formar CO 2 molécula (9) e (10). A reação entre Pt – CO anúncios e Ru– OHads leva a CO 2 evolução, gerando sites Pt e Ru atualizados (reação 10). Considerando que, outro trabalho feito por Ewelina Urbanczyk et al. [48] realizaram a reação de oxidação do metanol para o catalisador PtNi em meio alcalino (1,0 M KOH). Teoricamente, a reação de oxidação do metanol em meio alcalino é:
$$ {\ mathrm {CH}} _ 3 \ mathrm {OH} +6 \ mathrm {OH} \ to {\ mathrm {CO}} _ 2 + 5 {\ mathrm {H}} _ 2 \ mathrm {O} +6 { \ mathrm {e}} ^ {\ hbox {-}} $$
A reação inicia no eletrodo de Pt do DMFC para o dióxido de carbono. Durante esse processo, podem se formar moléculas intermediárias (CO) que podem causar envenenamento e desativação do lado ativo da Pt. Esta molécula de CO é um produto da oxidação incompleta do metanol. A oxidação incompleta do metanol forma o CO como o produto intermediário (Eq. 11). A superfície do eletrocatalisador também pode adsorver grupos hidroxila (Eq. 6). Por fim, devido à dessorção do produto principal, forma-se o gás carbônico (13). O segundo veneno que pode ser produzido durante a oxidação do metanol é o metano. Nesse caso, pode ocorrer a seguinte reação (8). A oxidação total da forma intermediária de carbono em dióxido de carbono na reação eletroquímica é a seguinte:
$$ 3 \ mathrm {Pt} + {\ mathrm {CH}} _ 3 \ mathrm {OH} \ to \ mathrm {Pt} - \ mathrm {COads} +4 {H} ^ {+} + 2 \ mathrm {Pt } + 4e - + {H} ^ 2O $$ (11) $$ \ mathrm {Ni} + {H} _2O \ to \ mathrm {Ni} - \ mathrm {OHads} + {H} ^ {+} + e - $$ (12) $$ \ mathrm {Pt} - {\ mathrm {CO}} _ {\ mathrm {ads}} + \ mathrm {Ni} - \ mathrm {OHads} \ to {\ mathrm {CO}} _2 + {H} ^ {+} + \ mathrm {Pt} + \ mathrm {Ni} + e- $$ (13) $$ \ mathrm {Pt} - {\ mathrm {CH}} _ 3+ \ mathrm {Pt} - H \ to 2 \ \ mathrm {Pt} + {\ mathrm {CH}} _ 4 $$ (14) $$ \ mathrm {Pt} - {\ mathrm {CH}} _ 3+ \ mathrm {Ni} {\ left (\ mathrm {OH} \ right)} _ 2 \ to \ mathrm {Pt} + {\ mathrm {CO}} _ 2+ \ mathrm {Ni} +5 {H} ^ {+} + 5e- $$ (15)
Atualmente, os pesquisadores ainda estão estudando técnicas de liga para melhorar a atividade catalítica de eletrocatalisadores à base de Pt pela fabricação de ligas ternárias e quaternárias de Pt, como PtRuSn [91, 92], PtRuNi [93,94,95], PtRuMo [70, 96 , 97], quaternário PtRuOsIr [79, 80] e PtRuIrSn [97, 98], devido ao seu excelente comportamento em MOR e remoção das espécies intermediárias (CO) que se formam na superfície de Pt. Mas, a adição do terceiro e quarto metais nesses catalisadores ternários e quaternários ainda é desconhecida. Além disso, existem algumas limitações e desafios na produção das ligas ternárias e quaternárias. A otimização da morfologia do catalisador e das composições do catalisador torna-se difícil de ser obtida por causa de muitas combinações possíveis de metais e composições. No entanto, muitos estudos provaram que a adição do terceiro e quarto metal aumentava notavelmente a atividade catalítica, aumentava a estabilidade do catalisador e tinha boa tolerância ao CO em relação à eletrooxidação do metanol e aplicações de DMFC.
Tsiouvaras et al. [99] conduziram a medição eletroquímica dos catalisadores PtRuMo / C e descobriram que embora todos os catalisadores ternários fossem mais ativos para a oxidação de CO e metanol do que o catalisador binário, o catalisador tratado com H 2 mostrou um desempenho melhorado em aproximadamente 15% em relação aos catalisadores ternários tratados em He ou sem tratamento. Em 2012, Hu et al. [100] sintetizou com sucesso um eletrocatalisador bimetálico superior (PtNi), nomeadamente nanoesferas ocas mesoporosas de PtNi (HMPNNs). O catalisador exibiu excelente desempenho catalítico no MOR com eficiência de utilização de Pt significativamente aprimorada devido à estrutura única de HMPNNs e sua grande área de superfície eletroquímica. Por volta de 2016, o trabalho feito por Yang et al. [101] também investigaram a reatividade de eletrocatalisadores bimetálicos de PtFe sintetizados nos quais observaram que a forte interação entre Pt e metais de ferro (Fe) pode diminuir as energias de adsorção de NPs bimetálicos. Eles também descobriram que as nanopartículas bimetálicas de PtFe preferem ser adsorvidas no grafeno de vacância única através dos átomos de Fe quando os átomos de Pt e Fe estão ambos nas superfícies, porque as interações entre os átomos de Fe e o grafeno de vacância única é mais forte do que aquelas entre os átomos de Pt e grafeno para uma única vaga. A Figura 3 ilustra a posição das partículas de Pt e Fe dispersas no suporte de grafeno, conforme sugerido por Yang et al. [101].
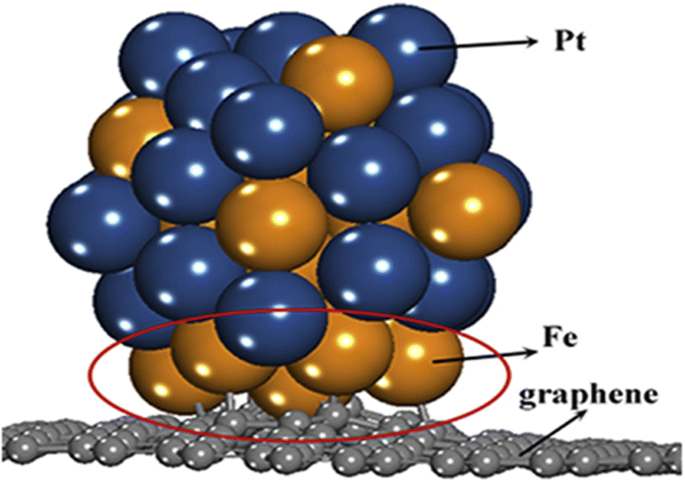
A posição do catalisador PtFe no suporte de grafeno ilustrado por Yang et al. [101]
Desempenho de catalisador baseado em Pt e carboneto de metal de transição
Carboneto de metal de transição (TMC), com alta estabilidade mecânica e química contra corrosão, boa resistência a ambientes ácidos, estabilidade de longo prazo e alta tolerância ao CO, pode atuar como catalisadores anódicos [88,89,90, 102,103,104]. Além disso, TMCs também fornecem muitas vantagens em comparação com seus metais originais no que diz respeito à atividade, seletividade e resistência a venenos, por exemplo, carboneto de tungstênio (WC) mostra propriedades especiais, como boa condutividade elétrica, resistência a ambientes ácidos, baixo custo e tolerância ao envenenamento por CO no processo de eletrooxidação do metanol [88, 105, 106].
Wang et al. [103] relatou a síntese de alta área de superfície (256 m 2 g −1 ) microesferas de carboneto de tungstênio por meio de um método hidrotérmico simples. W 2 C foi encontrado como a fase principal na amostra sintetizada. Atualmente, os pesquisadores estão explorando o potencial de Pt suportado em WC como um catalisador ideal para DMFC [38, 88, 107, 108]. Christian et al. [106] concluiu que em relação aos seus elementos de metal de transição, TMCs se comportam como metais nobres, como Pt, Pd, Rh e Ru para certas reações químicas e eletroquímicas, incluindo reação de oxidação de hidrogênio, monóxido de carbono e álcool e redução de oxigênio [109, 110]. Em outro estudo, Liu et al. [107] apresentaram que os carbonetos de molibdênio (Mo-Carbides) poderiam atuar como promotores para o carboneto de tungstênio e aumentar a atividade eletrocatalítica no DMFC. No entanto, sem a incorporação do metal Pt, a atividade eletrocatalítica do WC puro em relação ao MOR para um sistema DMFC ainda é baixa. Portanto, uma pequena quantidade de metal de platina adicionada ao componente WC é muito conveniente para obter a vantagem do efeito sinérgico entre Pt e WC [91, 111, 112]. Enquanto isso, Hassan et al. [109] revelou que a impureza comum (espécies de CO) que se forma durante a oxidação do metanol tem forte energia de ligação na superfície Pt; portanto, deve ser oxidado para que possa ser removido dos locais ativos de Pt. A adição do componente WC no eletrocatalisador Pt / WC mostrou alta tolerância ao CO para MOR, o que indica a existência de efeitos sinérgicos entre o metal Pt e WC como componente de suporte. O estudo também foi realizado por outro pesquisador usando menos metal Pt para reduzir o custo de Pt, mantendo um bom desempenho eletrocatalítico.
Fora isso, o componente WC é mais ativo para a formação de um grupo metoxi (CH 3 O-) do que Pt puro [113, 114]. O desempenho CV de (Pt:Ru) 4-WC / RGO mostra excelente desempenho catalítico com uma densidade de corrente de 330,11 mA mg −1 Pt em comparação com os outros cinco catalisadores, indicando que o eletrocatalisador sintetizado tem excelente atividade catalítica em relação ao MOR. Além disso, a combinação de Ru e WC no catalisador de Pt aumentou a quantidade de OH na superfície e permitiu que o CO adsorvido na superfície de Pt fosse oxidado em potenciais mais baixos [39].
Desempenho do catalisador à base de Pt e nitreto de metal de transição
O nitreto de metal de transição (TMN) é um candidato ideal como suporte do catalisador Pt devido à sua boa condutividade elétrica (metálica), dureza, alta estabilidade eletroquímica e resistência à corrosão em condições de operação de célula a combustível [115,116,117,118]. Nitretos de metais de transição, como CrsN, TiN e VN, catalisador de Pt com suporte foram relatados e mostraram alto desempenho catalítico e melhor estabilidade em comparação com os suportes de carbono tradicionais [112]. Todos os metais de transição podem formar nitreto, exceto a segunda e a terceira linha dos metais do grupo 8, 9 e 10 (Ru, Os, Rh, Ir, Pd e Pt). O comportamento e as propriedades estruturais dos nitretos de metais de transição podem ser encontrados na literatura [92,93,94]. Xiao et al. [112] prepararam um eletrocatalisador de Pt suportado por nitreto de cobalto e titânio que apresentou excelente desempenho e estabilidade em relação à reação de redução de oxigênio (ORR). O Ti 0,9 Co 0.1 O catalisador de Pt suportado em N exibiu um tamanho de partícula pequeno e boa dispersão de metal. Este eletrocatalisador preparado também manteve a área de superfície eletroquímica (ECSA) de Pt e melhorou muito a preservação de ECSA, com apenas uma diminuição de 35% na queda inicial de ECSA após 10.000 ciclos de ADT. A dopagem com cobalto aumentou significativamente a atividade e durabilidade da ORR. Enquanto isso, um eletrocatalisador durável e de alto desempenho no sistema DMFC pode ser obtido usando eletrocatalisador de grande área de superfície Pt (Ru) / TiN, que também demonstrou alta atividade eletroquímica para MOR com uma melhoria de ∼ 52% da atividade catalítica e boa estabilidade / durabilidade em comparação ao JM-Pt comercial (Ru). Enquanto isso, o desempenho de célula única do DMFC alcançou melhor densidade de potência máxima em 56% e demonstrou excelente estabilidade eletroquímica para o eletrocatalisador CSG-Pt (Ru) / TiN [115].
A pesquisa atual em nanopartículas de Pt suportadas em nanotubos de nitreto de ferro de titânio com estrutura oca e porosa e alta área superficial foi sintetizada por Li et al. [116]. Exibiu um aumento significativo na atividade eletrocatalítica em relação ao MOR em condição ácida e teve melhor durabilidade. Os motivos para essas propriedades foram devido aos seus dados experimentais de trabalho que verificaram que a adição de Fe pode sintonizar a estrutura eletrônica dos átomos de Pt, o que contribui para o fortalecimento da atividade e estabilidade do catalisador de Pt para o MOR. Enquanto isso, em trabalho anterior feito por Xiao et al. [117], Pt / Ti 0,8 Mo 0.2 O catalisador N exibiu uma estrutura porosa e alta área superficial, nanopartículas de Pt de tamanho pequeno e bem dispersas. Este sistema de catalisador preservou a estabilidade eletroquímica intrínseca da nanoestrutura de TiN e aumentou notavelmente a atividade e durabilidade de MOR. No entanto, as informações atualmente disponíveis sobre a estabilidade eletroquímica do nitreto de tungstênio (WN) ainda são insuficientes [109].
Enquanto isso, MoxN ( x =1 ou 2) no substrato de Ti exibiu estabilidade eletroquímica em um eletrólito ácido de 4,4 M H 2 SO 4 até potencial anódico de + 0,67 V (vs. SHE) ao longo de 50 ciclos repetidos [110]. No entanto, este eletrocatalisador apresentou danos de superfície, como rachaduras e desintegração, nas regiões de alto potencial catódico (abaixo de - 0,1 V vs. SHE) e anódico (acima de + 0,67 V vs. SHE) devido à corrosão catódica e anódica, respectivamente. Na região de alto potencial anódico acima de + 0,67 V (vs. SHE), a composição do oxigênio aumentou devido à formação do óxido MoOx, que poderia causar a desativação. Esses resultados indicam que o MoxN reage com as espécies de oxigênio presentes no eletrólito aquoso e é instável acima de + 0,67 V (vs. SHE). Mustafha et al. [111] descobriram que Pt carregado em TiN como um suporte mostrou eletroatividade para a oxidação do metanol, com uma alta razão If / Ib representando alta resistência de CO no voltamograma realizado a uma taxa de varredura de 20 mV / s em 0,5 M CH 3 OH + 0,5 M H 2 SO 4 como o eletrólito. O efeito bifuncional entre Pt e TiN foi citado como a causa da resistência ao CO de Pt / TiN. Além disso, Ottakam Thotiyl et al. [91] obtiveram bons resultados para um catalisador TiN carregado com Pt, mostrando tolerância ao CO muito boa para a oxidação eletroquímica do metanol. Eles concluíram que as características especiais do TiN que o tornam adequado como um suporte Pt para o MOR em um meio alcalino são que ele apresenta estabilidade excepcional, extrema resistência à corrosão, boa condutividade eletrônica e forte comportamento de adesão. Os catalisadores com suporte de TiN são benéficos em termos de estabilidade de longo prazo, densidade de corrente de troca e correntes estáveis em baixo potencial. Carregamentos de platina de 40% em peso em TiN foram usados nos experimentos.
Nos últimos anos, Liu et al. [118] sintetizou com sucesso platina em nitreto de níquel de titânio decorado com nanotubos de carbono 3D que reduziram o suporte de óxido de grafeno (TiNiN / CNT-rGO) por processo solvotérmico seguido por processo de nitretação. Pt com tamanho de partícula pequeno é bem disperso no suporte TiNiN / CNT-rGO. The 3D shape of CNT-rGO support gives a fast route for charge transfer and mass transfer as well as TiNiN NPs with good synergistic effect and the strong electronic coupling between different domains in TiNiN/CNT-rGO support. Thus, it greatly improved the catalytic activity of this catalyst. In another research, the non-carbon TiN nanotubes-supported Pt catalyst done by Xiao et al. [119] also displayed enhanced catalytic activity and durability toward MOR compared with the commercial Pt/C (E-TEK) catalyst.
Performance of Pt-Based Catalysts with Transition Metal Oxide
Pan et al. [92] reported the synthesis of platinum–antimony-doped tin oxide nanoparticles supported on carbon black (CB) as anode catalysts in DMFC, which exhibited better improvement in catalytic activity toward MOR compared to Pt-SnO2 /C or commercial Pt/C electrocatalyst. The enhancement in activity was attributed to the high electrical conductivity of Sb-doped SnO2 , which induced electronic effects with the Pt catalysts. Another work done by Abida et al. [93]described the preparation of Pt/TiO2 nanotube catalysts for methanol electrooxidation. O TiO 2 nanotubes-supported Pt catalyst (Pt/TiO2 nanotubes) exhibited excellent catalytic activity toward MOR and had good CO tolerance. They also reported that the use of hydrogenotitanate nanotubes as a substrate for the Pt catalyst considerably improved the COads oxidation on Pt, but the MOR still occurred at high potential. Then, several years later, Wu et al. [94] synthesized Pt-C/TiO2 with MOR activity 1.6 higher than commercial Pt-C and the stability of Pt-C/TiO2 was also enhanced by 6.7 times compared to Pt-C. The excellent performance of this catalyst was a contribution of mesopores and partially coated carbon support. Zhou et al. [95] prepared hollow mesoporous tungsten trioxide microspheres (HMTTS) using the spray-drying method to yield Pt/HMTTS. The electrocatalyst exhibited excellent electrocatalytic activity and high stability toward MOR than Pt/C and Pt/WO3 electrocatalysts, which may be attributed to the well-ordered Pt particles (with an average size of 5 nm) on the HMTTS surface. Wu et al. [120] used polystyrene spheres as templates to obtain pore-arrayed WO3 (p-WO3 ) The Pt nanoparticles with an approximate size of 3.3 nm dispersed on pore-arrayed WO3 (Pt/p-WO3 ) exhibited high catalytic activity toward MOR.
Li et al. [121] used Sn-doped TiO2 -modified carbon-supported Pt (Pt/Ti0.9 Sn0.1 O 2 –C) as an electrocatalyst for a DMFC system. The synthesized Pt/Ti0.9 Sn0.1 O 2 –C electrocatalyst revealed high catalytic activity and CO tolerance toward MOR. The enhanced catalyst activity was due to the high content of OH groups on the Ti0.9 Sn0.1 O 2 electrocatalyst sample and the strengthened metals and support interactions. In addition, Lv et al. [122] also reported in their work that the addition of TiO2 could not only facilitate CO removal and hinder CO formation on Pt surface during methanol oxidation, but it can also prevent the agglomeration and corrosion of Pt, which can be concluded from strong metal-supports interaction between TiO2 –C and Pt. Huang et al. [123] revealed that a TiO2 -coated carbon nanotube support for Pt electrocatalysts could be prepared via a one-step synthesis. Hao et al. [124] developed a new catalyst composed of Pt nanoparticles deposited on graphene with MoO3 . These catalysts exhibited high catalytic activity toward MOR and high resistance to CO species. However, the size of MoO3 must be tuned by controlling the metal oxide loading.
The selection of metal oxide such as MnO, RuO, CeO, SnO2 , MgO, and V2 O 5 as additional component in electrocatalyst of Pt because of their low cost, good electrochemical properties, and have proton-electron intercalation properties [125]. From the catalytic activity aspect, it can be summarized that the addition of these metal oxides can enhance the electrocatalytic activity of DMFC and other fuel cells. The incorporation of these conducting metal oxides together with Pt catalyst could also facilitate the oxidation process of CO intermediate molecules. Hence, these types of metal have high potential to be used together with platinum as anode electrode.
Carbon support
To improve the utilization of the Pt catalysts, the carbon support is also another useful approach to be used together with Pt. Carbon materials are largely used as catalyst support because of its special properties such as relatively stable in both acid and basic electrolyte, good conductivity, and provide high surface area for dispersion of metal catalyst. It is believed that carbon materials have a strong effect that can influence the electrocatalysts properties such as metal particle size, morphology, metal dispersion, alloyed degree, and stability. Carbon supports can also affect the performance of supported catalysts in fuel cells, such as mass transport and catalyst layer electronic conductivity, electrochemical active area, and metal nanoparticle stability during the operation.
Currently, a great concern of the development in the nanotechnology field, especially carbon nanomaterials synthesis, is to create more stable and active supported catalysts. Support materials of nanoparticles are believed to be the most promising materials for catalytic activity in fuel cells, including the DMFC system. Pt has been traditionally used as nobel catalysts for many fuel cells application [126,127,128]. However, the high cost and low reserve are hindering commercialization of fuel cells and driving researchers to make the utmost of the catalyst. According to this problem, the major effort has been done toward nanoscaling of the catalyst nanoparticles to form more active sites per mass unit. The morphology, structure, and activity of the catalyst, and correspondingly the whole lifetime of a cell, thus strongly depend on the catalyst support [129]. Table 2 shows the preparation, physical properties, performance, and activity of Pt-based supported carbon done by groups of researchers. The details of Pt-based supported carbon will be performed in the following sections:“Graphene Support” to “Carbon Nanocoils”.
Graphene support
Graphene has many extraordinary properties; it exists as a two-dimensional carbon (2-D) form, which is called a crystalline allotrope, one-atom-thick planar flat sheet of sp2 tightly bonded carbon atoms with a thickness of 0.34 nm. Its carbon atoms are packed in a regular atomic-scale chicken wire (hexagonal) pattern [92, 119]. The theoretical specific surface area of graphene is 2630 m 2 g −1 , which is much larger than that of carbon black (typically less than 900 m 2 g −1 ) and carbon nanotubes (100 to 1000 m 2 g −1 ) and similar to that of activated carbon [130]. Graphene has high potential as a metal support [131, 132, 133] [33] due to its high surface area [134] for better catalyst/metal dispersion [135], high electrical conductivity [136], and good thermal properties [137, 138]. Moreover, the functionality of graphene support can be modified by changing it surface structure, and hence contribute to its potential applications, such as in fuel cells, energy storage, electrochemistry, supercapacitors, and batteries [138,139,140,141,142]. Figure 4 illustrates the preparation steps to obtain the graphene nanosheets (GNS), while Fig. 5 shows their TEM images [143]. It can be clearly observed that the thickness of the GO with many typical wrinkles obviously decreases compared to graphite. This can be explained by the presence of the rich oxygen-containing functional groups over the surface of GO [132]. Besides, both resulting GN-900 and GN-900-C contained of a large size of nanosheets structure, but the GN-900-C comprised more transparent than the GN-900.
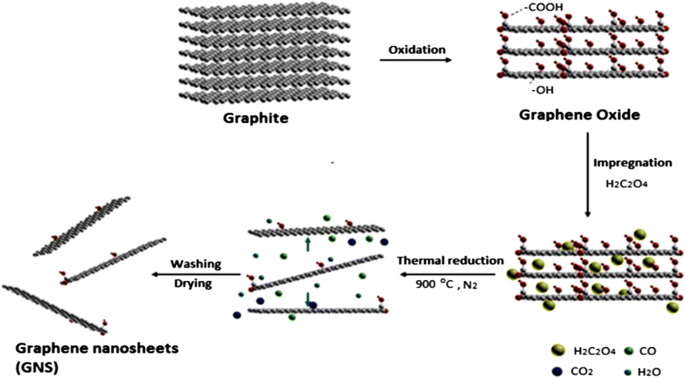
illustration of the preparation of graphite oxide to graphene nanosheets (GNS) by using oxalic acid [143]
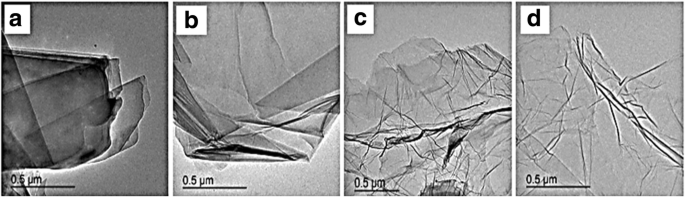
TEM images of graphite (a ), GO (b ), GN-900 (c ), and GN-900-C [143]
The discovery of graphene sheets began around 2000 by mechanical extracting process from 3D graphite source [133]. Graphene can be obtained by several synthesis methods such as hydrothermal [144], chemical reduction [143], chemical vapor deposition, and electrochemical. Ma et al. [145] enhanced the electrocatalytic activity of Pt nanoparticles by supporting the Pt nanoparticles on functionalized graphene for DMFC. Functionalized graphene was prepared by methyl viologen (MV) and Pt/MV–rGO electrocatalyst was synthesized by a facile wet chemical method. They also reported that the higher catalytic activity of Pt/MV–RGO was attributed to the synergetic effect between MV and rGO.
Meanwhile, Zhang et al. [146] modified the graphene support with graphene nanosheets through Hummer’s method, followed by polymerization of aniline (as nitrogen source). The TEM images for Pt/NCL-RGO and Pt/RGO electrocatalysts show that the aggregation between separated graphene sheets was decreased by nitrogen-doped carbon layer (NCL), leading to a better dispersion of the Pt catalyst on the graphene nanosheets support and better electroactivity and stability toward methanol electrooxidation (MOR). Presence of NCL successfully prevented the aggregation of graphene nanosheets as the Pt nanoparticles supporting material.
In 2011, Qiu et al. [135] successfully synthesized nanometer-sized Pt catalyst via sodium borohydride reduction method with an average particle size of only 4.6 nm. These Pt nanoparticles showed an even dispersion of Pt catalyst on graphene oxide support and very high electrocatalytic activity toward MOR by controlling the percent deposition of Pt loaded on the graphene. In another study conducted by Ojani et al. [147], for synthesized Pt-Co/graphene electrocatalyst, it was shown that graphene nanosheets improved the electrocatalytic behavior and long-term stability of the electrode. In addition, the Pt-Co/G/GC electrocatalyst showed great stability toward MOR. The catalytic performance toward MOR can also be improved by using cobalt core–platinum shell nanoparticles supported on surface functionalized graphene [148]. This enhanced catalytic activity could be attributed to the poly (diallyldimethylammonium chloride) (PDDA) that plays a crucial role for dispersion and stabilization of Co@Pt catalyst on graphene support. PDDA-functionalized graphene provided the higher electrochemical active surface area [149, 150]. Huang et al. [138] also studied a PtCo-graphene electrocatalyst with outstanding catalytic performance and high CO tolerance toward the MOR, which far outperformed Pt-graphene and PtCo-MWCNT electrocatalysts with the same ratio of Pt and carbon content. Figure 4 shows the formation of a graphene-PtCo catalyst prepared from a graphite source. Sharma et al. [57] synthesized Pt/reduced graphene oxide (Pt/RGO) electrocatalyst using a microwave-assisted polyol process, which sped up the reduction of GO and formation of Pt nanocrystals. They compared Pt/RGO to a commercial carbon support (Pt/C), which exhibited high CO tolerance, high electrochemically active surface area, and high electrocatalytic activity for the MOR. In a previous study, Zhao et al. [139] reported that the unique 3D-structured Pt/C/graphene aerogel (Pt/C/GA) exhibited greater stability toward MOR with no decrease in electrocatalytic activity. Moreover, the Pt/C/graphene aerogel also exhibited significantly higher stability to scavenge crossover methanol at high potential in an acidic solution compared with the commercial Pt/C electrocatalyst. At the initial catalytic stage, the Pt/C electrocatalyst lost approximately 40% after 1000 CV cycles. In contrast, the Pt/C/graphene aerogel only lost 16% of the initial catalytic activity. After 200 cycles of CV, the current density of Pt/C/graphene aerogel was much higher with a remarkably higher stability than that of Pt/C electrocatalyst. Meanwhile, Yan et al. [151] demonstrated highly active mesoporous graphene-like nanobowls supported Pt catalyst with high surface area of 1091 m 2 g −1 , high pore volume of 2.7 cm 3 g −1 , and average pore diameter of 9.8 nm obtained by applying a template synthesis method. In addition, the Pt/graphene bowls also achieved high performance toward MOR with a current density value of 2075 mA mgPt −1 , which was 2.87 times higher than that of commercial Pt/C (723 mA mgPt −1 ) The onset potential for the Pt/graphene bowls toward methanol electrooxidation was negatively shifted by approximately 160 mV compared with that to the latter and showed CO resistance. Figure 6 shows the proposed schematic for the formation of PtCo catalyst on reduced-GO (rGO) support [51]. It is described that the formation of graphene oxide nanosheets from oxidation of graphite powder leads to the increase in interlayer “d” spacing of stacked graphitic sheets from 0.34 to 0.78 nm due to the presence of various oxygen-containing functional groups. The oxygen-containing functional groups act as anchor sites for the well-dispersed Pt and PtCo nanoparticles on rGO sheets, and used for efficient electrooxidation of methanol.
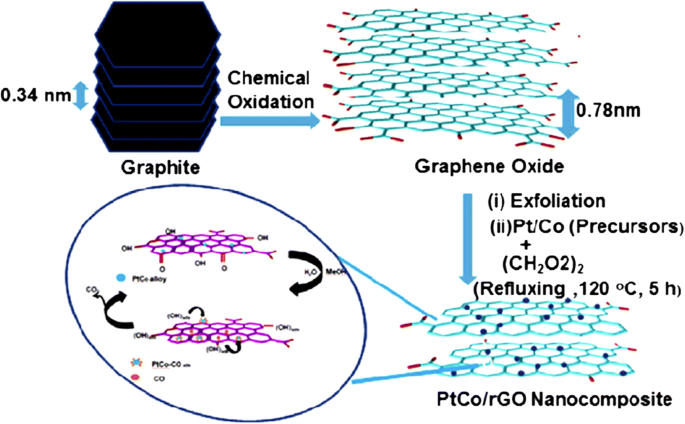
Illustrates the schematic formation of graphene supported Pt-Co catalyst [51]
We can conclude that the reduce graphene oxide (rGO), graphene, modified graphene as supporting material exhibited high electrocatalytic activity toward methanol electrooxidation process. A lot of studies have been reported related to the particle size distribution and size, morphologies, and catalytic activities of Pt and Pt alloys using graphene as supporting material, which showed great improvement in fuel cell performance as mentioned and discussed above. Thus, graphene support can be further studied for better fuel cell performance.
Multiwall Carbon Nanotube and Single-Wall Carbon Nanotube Support
Several years ago, Jha et al. [140] prepared multiwall carbon nanotube (MWCNTs) via chemical vapor deposition using an AB3 alloy hydride catalyst. Platinum-supported MWCNT (Pt/MWCNT) and platinum-ruthenium-supported MWCNT (Pt-Ru/MWCNT) electrocatalysts were prepared by chemical reduction. The performance of these electrodes was studied at different temperatures, and the results demonstrated a very high power density of 39.3 mW cm −2 at a current density of 130 mA cm −2 , which could be attributed to the dispersion and accessibility of the MWCNT support and Pt-Ru in the electrocatalyst mixture for the methanol oxidation reaction. This was also done by other researchers that using different catalyst supported MWCNT for DMFC system [152,153,154,155]. Meanwhile, Wu and Xu [156] compared MWCNT-supported Pt and single-wall carbon nanotube (SWCNT)-supported Pt. Figure 7 shows that the TEM images of Pt catalyst was deposited on MWNT and SWNT electrodes through the electrodeposition technique. The Pt particles in Pt-SWNT (Fig. 7b) looked closer contact with the network of entangled and branched bundles of SWNT support, and the shape is closer to highly exposed sphere. The benefits of the SWCNT support are due to its greater electrochemical surface-active area and easier charge transfer at the electrode/electrolyte interface because of the graphitic crystallinity structure, rich amount of oxygen-containing surface functional groups, and highly mesoporous and unique 3D-structure of SWNT. The electrodeposition technique carried out by them contributed to higher utilization and more uniform dispersion of Pt particles on its support.
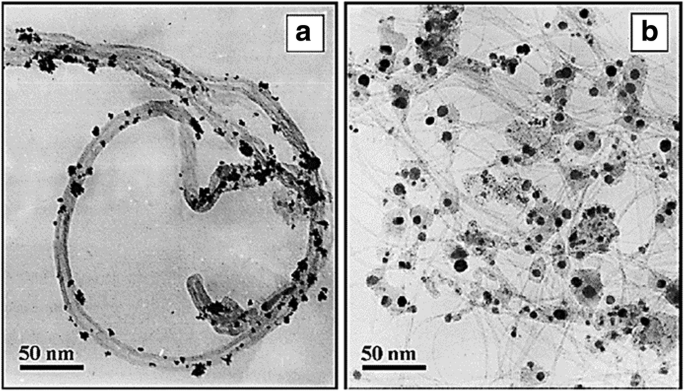
TEM images for the Pt on MWCNT(a ) and SWCNT (b ) [156]
Then, Wang et al. [157] reported the high performance of modified PtAu/MWCNT@TiO2 electrocatalyst prepared via deposition-UV-photoreduction for DMFC, which also exhibited high CO tolerance. Zhao et al. [126] studied 3D flower-like platinum-ruthenium (PtRu) and platinum-ruthenium-nickel (PtRuNi) alloy nanoparticle clusters on MWCNTs prepared via a three-step process, and the best ratios obtained from their experiments for the PtRu and PtRuNi alloys were 8:2 and 8:1:1, respectively. Another group, i.e., Zhao et al. [158], found a higher current density toward MOR and better activity for MWCNT-supported PtWC compared with Pt/C electrocatalyst. These results were attributed to the factors of the synergistic effect between the Pt catalyst and the WC component, high CO tolerance from the bifunctional effect of the Pt catalyst and the WC component, and strong interaction between metals and WC in the electrocatalyst composite.
As a summary, both of MWCNT and SWNT support in terms of structural, surface, and electrochemical properties have their own characteristics as supporting material that remarkably enhanced their performance in catalysis of methanol oxidation process. However, as a comparison, SWCNT possess a high degree of graphitization, highly mesoporous 3D structure, and contain more oxygen-containing functional groups at its surface sites. In relation with these properties, the SWCNT exhibits a higher electrochemically accessible surface area and faster charge transfer rate at the electrode/electrolyte interface.
Carbon Nanotube Support
Wen et al. [144] proposed that carbon nanotubes (CNTs) support could improve fuel cell performance; for example, Pt can be fixed to the inner wall and the outer wall of CNTs and may cause improvement in the electrocatalytic properties of platinum-CNTs. Yoshitake et al. [159] proposed that fuel cells using CNTs as the catalyst support produced larger current densities. The addition of binary or other components to the electrocatalysts for methanol electrooxidation overcomes the problems related to catalyst poisoning caused by CO during the reaction. Therefore, new electrocatalyst carbon supports, such as carbon nanotubes [160, 161], are being actively developed to significantly improve fuel cell performance. Kakati et al. [128] successfully synthesis the PtRu on CNT/SnO2 for anode catalyst DMFC via hydrothermal process. It has been found that the presence of SnO2 provide a high durability property for the catalyst and the presence of SnO2 in the district of Pt could supply oxygen-containing functional groups for the removal of CO intermediate molecules from the Pt surface sites during electrooxidation of methanol. Generally, the decomposition methanol occurs at Pt surface sites; meanwhile, the decomposition of water occurs at SnO2 surface sites to form oxygen-containing species which then react with CO intermediate molecules. However, as support material, the conductivity property of SnO2 still needs to be enhanced. Kakati et al. [128] also proposed the schematic diagram of the formation of PtRu on CNT/SnO2 composite as shows in Fig. 8, and FESEM images of CNT/SnO2 composite support (a and b) and PtRu/SnO2 /CNT composite electrocatalyst (c and d) in Fig. 9.
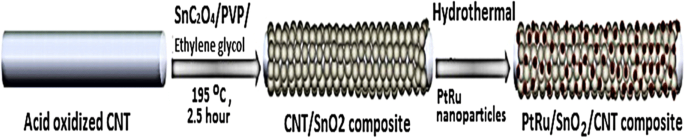
Illustrates the schematic diagram for the formation of PtRu/SnO2/CNT composite [128]
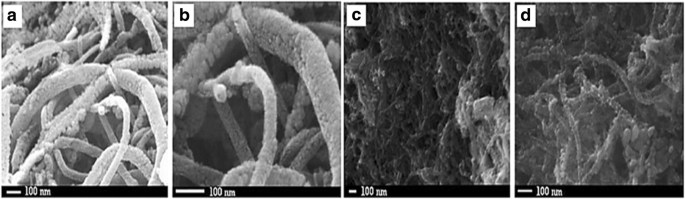
FESEM images of CNT/SnO2 composite support (a , b ) and PtRu/SnO2/CNT composite electrocatalyst (c , d )
Chien et al. [127] proposed that the high catalytic performance of Pt-Ru/CNT for MOR can be attributed to the presence of CNT as the carbon support material with several factors:(i) the as-synthesized Pt-Ru/CNT electrocatalyst owns the ideal nanosized particles and composition to increase catalytic activity, (ii) the presence of functional group on the CNT surface results in high hydrophilicity of CNT, which produces better electrochemical reaction on the electrode area, and (iii) the high electronic conductivity of the CNT support lowers the resistance in MOR. Jeng et al. [150] prepared Pt-Ru/CNT electrocatalyst via a modified polyol with a PtRu composition ratio of 1:1, exhibiting high catalytic activity toward MOR and better performance than that of commercial PtRu/C. Show et al. [162] reported that Pt catalyst with a size of less than 10 nm can be obtained by dispersing the Pt particles on a CNT surface using the in-liquid plasma method, and excellent performance was demonstrated by the electrical power achieving 108 mW cm −2 [162]. The in-liquid plasma method was also used by Matsuda et al. [163] that can applied to obtain nanometer-sized Pt catalyst on support material that remarkably enhanced the fuel cell performance.
To be concluded, high electric conductivity, large surface area, excellent chemical and electrochemical stabilities, quasi one-dimensional structure, and good morphology as the supporting materials are the key factors of carbon nanotubes (CNTs) in enhancing the DMFC performance. In addition, carbon support materials such as CNTs which contribute a large effect on metal distribution and size have also been proven to be an essential to the electrocatalysts to achieve high catalytic activity during methanol oxidation process.
Carbon Nanofiber Support
Steigerwalt et al. [164] reported the successful synthesis of PtRu alloy that was widely dispersed on a graphene carbon nanofiber (CNF) support as an electrocatalyst in DMFC. The catalytic activity was enhanced by ~ 50% relative to that recorded for an unsupported PtRu colloid anode electrocatalyst. Meanwhile, Wang et al. [152] reported that Pt/CNF nanocomposites obtained by the reduction of hexachloroplatinic acid (H2 PtCl 6 ) precursor with formic acid (HCOOH) in aqueous solution containing electrospun CNFs at room temperature showed a higher current density than other prepared Pt/CNFs and was approximately 3.5 times greater than that of the E-TEK Pt/C electrocatalyst. Another research carried out by Giorgi et al. [153] described a CNF and bimetallic PtAu electrode with a single layer and both diffusive and catalytic functions using a decreased noble metal amount (approximately five times less) with a consequent large cost reduction. In addition, the bifunctional electrocatalytic properties were also active for the MOR on the PtAu nanoparticle catalysts [154]. Calderón et al. [155] reported PtRu/CNF prepared via reduction using sodium borohydride (NaBH4 ), methanol, and formate ions. This electrocatalyst synthesized by SFM was heat-treated (denoted as SFM TT), which improved its electrocatalytic activity during MOR. Later, Maiyalagan [165] reported that the addition of silicotungstic acid acted as a stabilizer for the PtRu particles on CNT support. The PtRu-supported CNT was prepared by microwave heating of an ethylene glycol (EG) solution of STA, H2 PtCl 6 .6H 2 O (as Pt precursor), and RuCl3 .xH2 O (as Ru precursor) with CNF suspended in the solution. The Pt and Ru precursors were loaded on CNF by conventional impregnation method. The results revealed that the PtRu nanoparticles are uniformly dispersed on carbon nanofiber support, with an average particle size of 3.9 nm enhanced the catalytic activity toward methanol electrooxidation. As a conclusion, the carbon nanotubes supporting material with high electronic conductivity and high surface area gives an advantage of better dispersion for the Pt or Pt alloys deposition. The higher the surface area of supporting material can reduce the agglomeration of metal particles on it, thus can produce better catalyst morphology for better fuel cell performance.
Mesoporous Carbon Support
Mesoporous carbon (MPC) support is another ideal candidate as an electrocatalyst support material in DMFC and fuel cell. Generally, mesoporous carbons are divided into two classes based on their structures which are ordered mesoporous carbons (OMCs), with highly ordered pore structure and uniform pore size, nonordered mesoporous carbons with irregular pores. Other than that, OPC can be produced by using high quality of SBA-15 silica and sucrose as carbon source template. To prepare the high quality of SBA-15 SBA-15 sample, triblock copolymer, EO20-PO70EO20 (Pluronic P123, BASF), as the surfactant and tetraethyl orthosilicate (TEOS, 98%, Acros) as the silica source are used, as reported by literature [166,167,168]. The synthesis of MPC starts from synthesis of SBA-15, followed by calcination process.
A well-dispersed and ultralow Pt catalyst (PtFe) supported on ordered mesoporous carbon (OMC) was prepared via a simple route and showed superior catalytic activity. The PtFe alloy with a size range of 3–5 nm was homogeneously dispersed on the CMS with a very high specific surface area of more than 1000 m 2 g −1 [169]. The incorporation of Fe was discussed in the previous section (“Performance of Various Types of Pt-Based Catalysts” section and “Performance of Pt-Based Alloys” section). The high specific surface area of mesoporous carbon support can be produced by carbonization process of a resorcinol-formaldehyde polymer with a cationic polyelectrolyte as a soft template [160]. The performance of Pt/MPC also related to the synthesis/preparation method as done by Kuppan and Selvam. Kuppan and Selvam [167] synthesized four type of Pt/mesoporous carbon by using different reducing agent which are NaBH4 , EG, hydrogen, and paraformaldehyde. From there, the Pt/mesoporous carbon synthesized using paraformaldehyde as reducing agent for showed highest current density. The highest in catalytic was attributed to the use of paraformaldehyde that gives the smallest Pt particle size (4.5 nm), and the highest ECSA (84 m 2 /g) belongs to Pt/mesoporous carbon.
Wang et al. [161] synthesized a Pt@WC/OMC electrocatalyst composite, in which the composite was platinized using a pulsed microwave-assisted polyol technique. The OMC produced in this synthesis exhibited high surface area property. The Pt@WC/OMC electrocatalyst also showed high activity, desirable stability, and CO tolerance toward MOR. In another work done by Zhang et al. [170], the ordered CMS had a unique hierarchical nanostructure (with a 3-D structure) with ordered large mesopores and macropores that facilitated the dispersion of Pt nanoparticles and rapid mass transport during the reactions.
To maximize the use of Pt particles, the support materials should have uniform dispersion, high utilization efficiency, and desirable activity and stability. Moreover, the good supporting materials must be suitable for surface chemistry, high loading of Pt dispersion, and some functional roles. Additionally, based on the previous studies as discussed above, the ordered mesoporous carbons with large pore sizes are highly desirable for fast mass transfer and, thus, enhance the catalytic activity especially in the reaction involve large reactants molecules.
Carbon Black
Carbon black (CB) is one of the commercial carbon support that has been used till now. There are many types of CB such as Vulcan XC-72, Black Pearl 2000, Denka Black, Shawinigan Black, Ketjen EC-300J, etc. [171, 172]. CB is commonly used as a carbon support material for electrocatalysts because it possesses high porosity properties, which make it suitable as a potential support material for the catalyst layer in PEMFCs and DMFCs as reported in provided literatures [173,174,175,176,177,178,179,180]. The comparison of the several carbon black support was reported by Wang et al. [181] who investigated the effect on DMFC performance using several types of carbon black such as Vulcan XC-72R, Ketjen Black EC 300J, and Black Pearls 2000 carbon black as additives/support for the Pt cathode catalyst. From the experiments, the results showed that Ketjen Black EC 300J was the most useful carbon support for increasing the electrochemical surface area and DMFC performance of the cathode catalyst.
Nowadays, CB is commercial carbon support for many fuel cell systems. Generally, it is used for the comparison with new or modified catalyst [125]. The following Table 3 summarizes the commercial carbon black and its properties for fuel cell application. There are so many modifications among carbon support materials and development of new carbon support for enhance fuel cell performance; however, commercial carbon black still is used in many fuel cell applications especially for the comparison with new or modified catalyst.
Carbon Nanocoils
Celorrio et al. [182] proposed carbon nanocoils (CNCs) as a PtRu support in their experiment, indicating that the electrocatalyst performance was strongly dependent on the synthesis method. CNC-supported electrocatalysts showed better electrochemical behavior than E-TEK electrocatalysts, and better electrocatalytic behaviors toward CO and methanol oxidation were achieved using CNC as a support material [182]. Sevilla et al. obtained highly graphitic CNCs from the catalytic graphitization of carbon spherules via the hydrothermal treatment of different saccharides which are sucrose, glucose, and starch [183]. They demonstrated that the high electrocatalytic activity of the CNCs is due to the combination of good electrical conductivity of their graphitic structure and high porosity property, which allows much less diffusional resistance of reactants/products. Two years later, Sevilla et al. [184] reported highly dispersed Pt nanoparticles on graphitic CNCs with diameters in the range of 3.0–3.3 nm and a very fine particle size distribution. The electrocatalyst possessed large active Pt surface area (up to 85 m 2 g −1 Pt), high catalytic activity toward MOR (up to 201 A g −1 Pt), and high resistance against oxidation, which was noticeably greater than that of the Pt/Vulcan electrocatalyst. Celorrio et al. [185] obtained Pt/CNC electrocatalysts via the impregnation method, which showed that a combination of Pt and CNCs facilitated the CO oxidation process.
Conductive Polymer Supports
Choi et al. [186] synthesized PtRu alloy nanoparticles with two types of conducting polymers, i.e., poly(N -vinyl carbazole) and poly(9-(4-vinyl-phenyl)carbazole), as the anode electrodes. Electrochemical and DMFC tests showed that these nanocomposite electrocatalysts were beneficial in a DMFC system, but their catalytic performance was still lower than that of a carbon supported electrode. Thus, they suggested that higher electrical conductivity of the polymer and lower catalyst loss are required in nanocomposite electrodes to achieve better performance in a DMFC. Choi et al. [171] and Kim et al. [172] prepared polyaniline (PANi) as a support material for PtRu catalyst in a DMFC system. PANi is a group of conductive polymers with high electronic conductivity and a methanol oxidation current similar to that of carbon-supported PtRu catalyst. Then, Kim et al. [172] conducted catalytic tests to compare PtRu/PANi support with PtRu/carbon support, showing that the enhanced catalytic activity of PtRu/PANi was due to (i) the high electrical conductivity of the polyaniline support, (ii) the increase of electrochemical surface area of the prepared electrocatalyst, and (iii) the higher ion diffusion behavior. In another study, Amani et al. [74] synthesized PtSn supported by C-PANI as an electrocatalyst with different Pt:Sn atomic ratios using the impregnation method. The PtSn/C-PANI electrocatalyst with a ratio of 30:70 showed outstanding performance in the methanol electrooxidation, and the current density was approximately 40% higher than PtRu/C and 50% higher than Pt/C-PANi. The CO tolerance and stability were improved compared to that of PtRu/C, and the methanol crossover was reduced. Yaldagard et al. [173] studied the electrocatalytic performance of Pt/PANi/WC/C electrocatalyst for methanol electrooxidation (MOR) and oxygen electro-reduction (ORR), and it exhibited higher MOR activity, high CO resistance, and improved stability compared to Pt/C electrocatalyst in the presence of methanol.
Wu et al. [174] presented polypyrrole nanowire networks (PPNNs) as the anodic microporous layers (MPLs) of passive DMFC. In passive DMFC system, the novel MPL achieved a 28.3% increase in the power density from 33.9 to 43.5 mW cm −2 compared with the conventional layer with a similar PtRu (1:1). The high performance was due to the presence of PPNNs, which expressively improved the catalyst utilization and mass transfer of methanol on the anode. Besides, Selvaraj and Alagar [175] prepared Pt-Ru nanoparticle-decorated polypyrrole/multiwalled carbon nanotubes (Ppy/CNT) via the in situ polymerization of Ppy on CNTs containing ammonium peroxydisulphate (NH4 ) S 2 O 8 as an oxidizing agent at the temperature range of 0–5 °C, followed by deposition of Pt particles on PPy-CNT composite films via chemical reduction to produce Pt/PPy-CNT. It was found that the PtRu particles deposited on PPy–CNT composite films exhibited higher catalytic activity and stability toward MOR compared to Pt/PPy-CNT. So far, the investigation on polymer as supporting materials is not much as carbon support materials. From aspect as supporting materials, the performance of polymer support was not good/excellent as carbon support. Further studies are needed in the future for better electrocatalytic activity and DMFC performance.
Problems and Limitations of Using Pt for DMFC Systems
There are two major challenges in the development of new DMFC catalysts:(i) performance, including the catalytic activity, reliability, and durability; and (ii) catalyst cost reduction. Two major problems arise in DMFC when using pure Pt alone as the anode catalysts:(1) slower kinetics oxidation of methanol, even on some state-of-the-art anode catalysts, and methanol crossover through the membrane, which not only lowers cathode performance but also reduces fuel efficiency. To develop successful fuel cell technology, including DMFC technology, new catalysts must be investigated to improve the performance and reduce the cost. Reduction of the catalyst cost remains a major challenge. Currently, platinum is one of the most effective electrocatalysts for DMFC due to its high catalytic activity for methanol oxidation, but because it is a precious metal, platinum usage is challenging and limited [176, 177]. Therefore, many scientists have attempted to find materials that can behave like Pt catalysts. One problem with the MOR in DMFCs is that CO is produced as an intermediate reaction product when using Pt catalyst and has strong binding energy on platinum particles, poisoning the active sites of the platinum surface area [58]. Therefore, CO must be removed by oxidizing it from the Pt surface using another material with high resistance to CO poisoning. For example, Hwu et al. proposed Pt-modified WC catalyst that has remarkable resistance to CO poisoning [178]. On the other hand, they also suggested that CO tolerance originates from the lower CO desorption temperature on pure and Pt-modified WC compared to pure Pt.
There are many solutions that can be applied to reduce the cost of Pt, overcome or minimize the formation of CO species during methanol oxidation, and increase the kinetics of methanol oxidation, such as alloying with other metals or transition metals, the incorporation of metals, metal nitrides, and metal oxides and the use of carbon supports as discussed in this paper. However, to overcome this problem, we need to understand the formation of CO on Pt sites particle, and understanding of the mechanism of the anode reaction in DMFCs. Unfortunately, it has limited amount of mechanistic insight to be studied, because this reactions involve complex mechanism path with many possible intermediate molecules and also competing reaction pathways [179]. For Pt catalytic mechanism, it has been suggested by a direct reaction path. Unfortunately, the use of Pt on other metals has limited mechanistic information available. Figure 10 represents the reaction path for methanol electrooxidation and their possible intermediates molecules formed during the process. Black arrows show direct path, while green arrows show the indirect mechanism for CO2 formation as a final product. In the direct mechanism, the reaction path does not involve a CO intermediate, and CO2 is formed directly from methanol. In contrast, indirect mechanism forming a CO intermediate molecule and subsequently it is oxidized to CO2 produtos. Notably, CO is the most stable molecule of all the intermediates on Pt during MOR. The stability of CO causes it to be a main reason for the extensive CO poisoning problem that is often found on Pt catalyst.
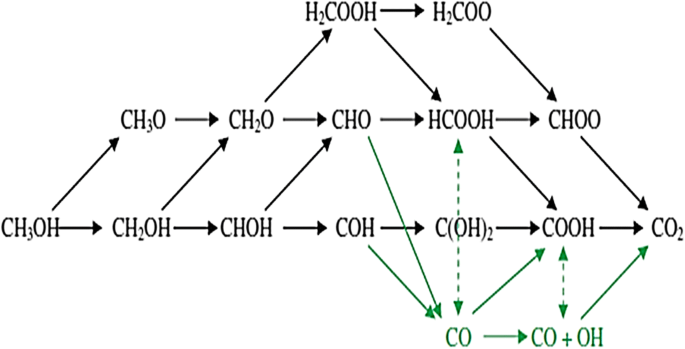
Schematic of the reaction paths and possible intermediates molecules considered in methanol electrooxidation [237]
First step in the mechanism of methanol decomposition reaction on Pt is the activation of methanol molecule. It can take place via hydrogen abstraction from either the carbon or the oxygen atoms. Further step, hydrogen abstraction creates formaldehyde (CH2 O) or hydroxymethylene (CHOH), followed by formyl (CHO) or COH. In the direct mechanism, instead of stripping off the final hydrogen from CHO or COH molecule to CO, a water molecule will release a proton/electron pair and resulting to OH species that can further bind with the carbonaceous species to form dihydroxycarbene (C(OH)2 ) or formic acid (HCOOH). This step is called hydroxyl addition process. The next step is followed by dehydrogenation to form either formate (HCOO) or carboxyl (COOH) molecule, with subsequent dehydrogenation to form CO2 as the final product of reaction. In addition, an alternative direct mechanism involve the stripping of a proton/electron pair from water and addition of the resulting hydroxyl to CH2 O, subsequently to H2 COOH, which then undergoes dehydrogenation to form HCOOH or dioxymethylene (Hs COO). The Hs COO molecule can then undergoes dehydrogenation to HCOO and finally to CO2 . Besides, in the indirect mechanism, CHO or COH species are directly dehydrogenated to CO. Water is dissociated separately on the surface to form OH, and the two surface species react together to form CO2 gas in a way similar to the water-gas-shift reaction [187]. This indirect mechanism occurs because less energy is required to form CO than CO2 . Strong adsorbed CO intermediate form on the Pt surface sites revealed a major problem at the anode site of DMFC. Formation of this intermediate species can cause deactivation Pt catalyst. Furthermore, the rate of kinetic methanol oxidation for DMFC is slower. Therefore, to increase the resistance of Pt catalyst to CO poisoning on the electrodes, Pt alloy or hybrids, such as PtRu, PtSn, PtMO, PtPb, PtFe, PtCo, PtNi, PtRuOs, PtRuMo, PtRuSn, PtRuNi, etc. (as mentioned and discussed in “Performance of various types of Pt-based catalysts” section), are usually employed as electrocatalyst materials on DMFC anodes. Addition/incorporation of these alloys to Pt can prevent the adoption of CO on Pt surface by decreasing the oxidation overpotential of the anode [84].
Conclusion and Prospects
Great progress has been made in recent years in the development and optimization of new catalysts using Pt-based catalysts and carbon and conductive polymer supports for DMFC anode catalyst. Some new carbon materials, such as nano- or mesostructured carbons, have been demonstrated as highly potential catalyst support materials, although their applications face challenges in terms of synthesis, metal loading, and electrode preparation. The combination of platinum as the best metal catalyst for DMFC and an excellent carbon support could produce breakthroughs in the investigation of a new DMFC anode catalyst in the future. Since platinum is an expensive metal, it is necessary to reduce the amount of Pt used in the electrocatalyst. Therefore, this paper presented more than 100 studies on the electrocatalytic activity and performance related to Pt-based electrocatalysts and various carbon and conductive polymer supports. The main problems related to the platinum electrocatalyst, such as carbon monoxide formation during the methanol oxidation reaction and the poor kinetics of methanol oxidation, could be overcome using additional materials and various supports, as reported in the research presented in this paper.
Many studies conducted in the recent years to reduce the loading amount of Pt catalyst and to increase the percentage utilization efficiency, and hence, enhance the electrocatalytic activity of Pt toward the oxygen reduction reaction (ORR) and methanol electrooxidation reaction (MOR), were discussed in this paper. Pt has been alloyed with many transition metals such as Fe, Co, Ni, Ir, Ru, Rh, and Pd, resulting in higher catalytic activity for the DMFC system. The incorporation of these materials also resulted in good dispersion on the carbon and polymer supports, which showed higher performance in the DMFC test compared to the use of Pt metal alone. Various carbon support sources, namely activated carbon (AC), carbon black (CB), multiwall carbon nanotubes (MWCNTs), carbon nanofibers (CNFs), carbon nanotubes (CNTs), graphene, and conductive polymer supports, have been used with Pt-based catalysts to improve their catalytic performance. Additionally, Pt-based alloy catalysts have been designed as hollow mesoporous PtNi, nanowire PtRu, and nanodendritic PtRh, which showed improved electrocatalytic activity and superior electrocatalytic performance. Meanwhile, 3-D Pt/C/graphene aerogel demonstrated enhanced stability toward methanol electrooxidation. The work performed by researchers showed that the electrocatalytic activities of nanoparticles Pt alloy catalysts depend on several factors such as the synthesis method, condition of experiments (such as temperature and pH), alloy composition/ratio, precursors, and thermal treatment. For the future study, it should be extended to the optimization of the geometry and structure of previous studies that revealed active Pt alloys can increase their electrocatalytic activity and stability and the application of support materials for fuel cell applications. For example, current research that have been done by Liu et al. 2017 [188] shows the excellent performance of platinum. From theoretical calculations, it revealed that the main effective sites on platinum single atom electrocatalysts are single-pyridinic-nitrogen-atom-anchored single-platinum-atom centers, which ascribed to the tolerant CO in MOR. They also suggested that carbon black supported used together with Pt single atom is effective in cost, efficient, and durable electrocatalyst for fuel cell application. According to the above study, herein, we can conclude that the modification on structure and morphology of precious metal such as platinum could also remarkably increase the performance of electrocatalyst, but in the same time can reduce the overall cost of fuel cell for commercialization.
To improve the morphologies of Pt and Pt alloys, carbon support material also need further study. Nanoporous metals become an interesting part of catalyst to be studied for fuel cell application. It is determined very suitable for fuel cell catalysts because they possess high surface area, three-dimensional (3D) network structures with adjustable ligament/pore sizes suitable for mass transport, and electron conduction. Around 2017, Li et al. successfully carried out modification on Pt-Pd-Au trimetallic surface as cathode for oxygen reduction reaction [189]. The surface evolution of 3-D Pt-Pd-Au trimetallic greatly enhanced the ORR activity and highly stable as ORR catalyst. The modification of PtNi alloy also done by Li et al. 2016 [190] showed ultrafine jagged platinum nanowire with highly large ECSA that exhibits enhanced mass activity of ~ 50 times higher than state-of-the-art commercial Pt/C catalyst, while Bu et al. 2016 [191] reported highly uniform PtPb/Pt core/shell nanoplate with biaxially strain extremely active, stable for anodic oxidation reactions, and great performance compared to commercial Pt/C in both methanol oxidation reaction (MOR) and ethanol oxidation reaction (EOR). Since the nanostructured platinum becomes an efficient catalyst for fuel cells as well as various industrial chemical reactions. Thus, these modifications on surface of Pt particles electrocatalysts could also to be applied in MOR for future DMFC.
On the other hand, to reduce the consumption of the Pt catalysts, the modification of the carbon support is also another useful way. This not only improves the transport capacity of protons but also reduces the usage of Nafion, which can cut the cost of the fuel cell. Moreover, with regards to the carbon support for the ORR catalysis, the hydrophobic carbon support material is required to allow water (product) to be quickly removed from the catalyst surface sites, and oxygen (reactant) to access the active sites. In contrast, the MOR catalysis requires a certain degree of hydrophilic carbon support. It can be achieved by the modification of the carbon support materials. By combination of modified carbon support materials and development of new carbon support with Pt metal catalyst, it is possible to get an ideal electrocatalysts for direct methanol fuel cell technology. Combination of Pt metal with varied carbon supports with different specific surface areas, structures, pore sizes, electronic properties, and morphologies could be great catalyst to be studied for future DMFC.
Carbon support also influence the overall performance for DMFC. Vulcan XC-72R, which is a commercial carbon support, has a large surface area, appropriate particle size, and good electrical conductivity for good support. However, in the process of depositing metal particle on these support with loading of 40% or more, the particle size of metal increased quickly, which is a disadvantage for DMFC, because a higher metal loading is used to give a better performance. In addition, multiwalled carbon nanotubes (MWCNTs) and carbon nanofibers (CNFs) with relatively smaller surface area, large diameter, and high aspect ratio could be very difficult to deposit a catalyst with high loading metal (40% and more). Therefore, modification of MWCNTs and CNFs support must be done to improve its surface area, surface functional groups, and reduce the wall thickness to achieve outstanding performance for direct methanol fuel cell even though high loading metal catalyst is consumed. As well, a great and important part to be further studied in DMFC system is about the anode and cathode catalyst preparation approaches.
Abreviações
- CB:
-
Carbon black
- CH 3 O:
-
methoxy group
- CNC:
-
Carbon nano cage
- CNF:
-
Carbon nano fiber
- CNT:
-
Carbon nano tube
- Co:
-
Cobalt
- Co:
-
Cobalt
- CO:
-
Monoxide molecules
- CO2 :
-
Carbon dioxide
- DMFC:
-
Direct methanol fuel cell
- FC:
-
Fuel cell
- Fe:
-
Ferro
- MOR:
-
Methanol oxidation reaction
- MPC:
-
Mesoporous carbon
- MWCNT:
-
Multi wall carbon nanotube
- Ni:
-
Níquel
- OMC:
-
Ordered mesoporous carbon
- ORR:
-
Reação de redução de oxigênio
- PANi:
-
Polianilina
- PEMFC:
-
Proton exchange membrane fuel cell
- Ppy:
-
Polypyrrole
- Pt:
-
Platina
- Pt/MWCNT:
-
Platinum-supported MWCNT
- Pt-Ru/MWCNT:
-
Platinum-ruthenium-supported MWCNT
- Rh:
-
Rhodium
- Ru:
-
Ruthenium
- Sn:
-
Sternum
- SOFC:
-
Solid oxide fuel cell
- SWCNT:
-
Single-wall carbon nanotube
- TMN:
-
Transition metal nitride
Nanomateriais
- Nanopartículas multifuncionais de ouro para aplicações diagnósticas e terapêuticas aprimoradas:uma revisão
- Avanços e desafios dos nanomateriais fluorescentes para aplicações biomédicas e de síntese
- Compostos de grafeno e polímero para aplicações de supercapacitor:uma revisão
- Fabricação e caracterização de novo composto de suporte de catalisador anódico de nanofibra de carbono Tio2 para célula de combustível de metanol direto via método de eletrofiação
- Desempenho aprimorado de um novo catalisador PdAu / VGCNF anódico para eletro-oxidação em uma célula de combustível de glicerol
- Avaliação de estruturas de grafeno / WO3 e grafeno / CeO x como eletrodos para aplicações de supercapacitor
- Novo suporte de catalisador anódico para célula de combustível de metanol direto:caracterizações e desempenho de célula única
- Aplicações Biomédicas para Nanoclusters de Ouro:Desenvolvimentos Recentes e Perspectivas Futuras
- Revisão:Filtros de Metal Poroso e Membranas para Separação Óleo-Água
- Solvay lança fita de fibra de carbono de alto desempenho para aplicações offshore de petróleo e gás